Objectives
The objective of our study was to develop a robust dual selection system used to identify PobR mutants responsive to a series of aromatic compounds. We first built a PobR mutant library that was established through random mutagenesis and included 21,000 mutants to evaluate the effectiveness of our dual selection system.
Results
Construction of biosensors
Based on the aforementioned design, we placed the mCherry (a red fluorescent protein) gene, cytosine deaminase (codA) gene, and chloramphenicol resistant (cmr) gene under the control of the pobR promoter (PpobA) to create the dual selection system (Figure 1). In the expression vector, the pobR gene is controlled by a constitutive promoter, and the genes responsible for the selection are driven by the PpobA. The consensus element (Oi) can bind PobR leading to repressed expression of the selective genes. When the PobRWT is allosterically activated by its native ligand 4HB and subsequently triggers the PpobA promoter, CDase is produced and converts exogenously added non-toxic 5-FC to toxic 5-FU, representing a negative selection. When the PpobA is triggered by a PobR mutant that is allosterically activated by a tested aromatic compound in the absence of 5-FC, the bacteria become Cm-resistant.
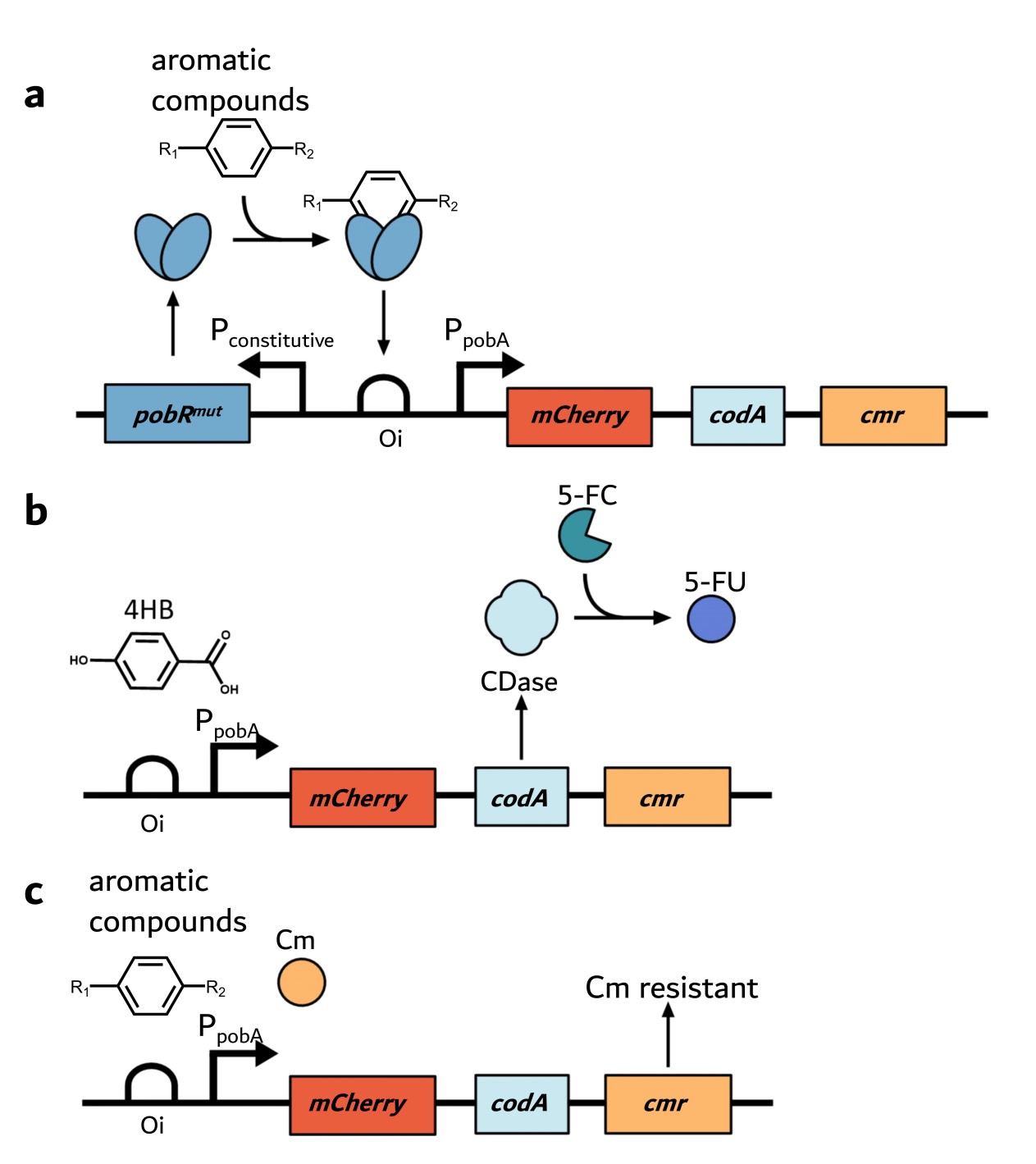
Figure 1. Schematic diagram of the dual selection system.
Thereafter, we verified the feasibility of the dual selection system using the expression vector containing gYB2a-pobRWT-mCherry-codA-cmr, the PobRWT biosensor. Before starting, we transferred the PobRWT biosensor into the E. coli BW25113 strain, in which the codA gene was knocked out (BWΔcodA). In the presence of 0.5 g/L 4HB, the PobRWT biosensor strain was resistant to chloramphenicol, and the growth of the bacteria was significantly better than that without 4HB. In experiments simulating the negative selection, the strains grew normally in the presence of either 4HB or 5-FC alone, but the bacterial growth was inhibited with both chemicals present in the medium (Figure 2).
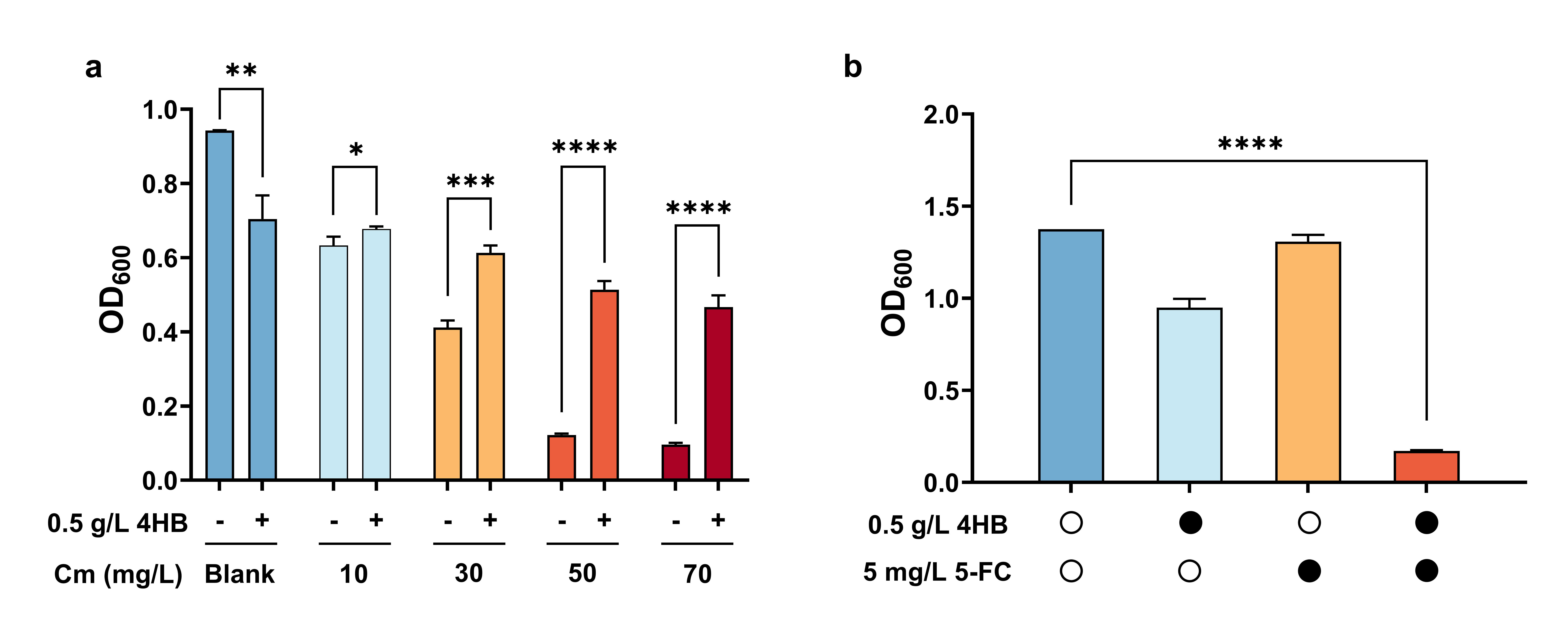
Figure 2. Model experiments of negative and positive selections using the dual selection system. The OD600 was measured after 12 h of cultivation.The criterion of statistical significance level was denoted as follows: *P < 0.05; **P < 0.01; ***P < 0.001.
Construction of mutant libraries
We designed primers with special interfaces, and obtained a large number of PobR mutant fragments by error-prone PCR, which were subsequently inserted into the vector harboring the PpobA, mCherry, codA and cmr, through the Golden Gate Assembly system, and finally transformed into the BWΔcodA strain. As a result, we constructed a random mutagenesis PobR library with a storage capacity of 21,000 clones and an average mutation rate of 0.36%.
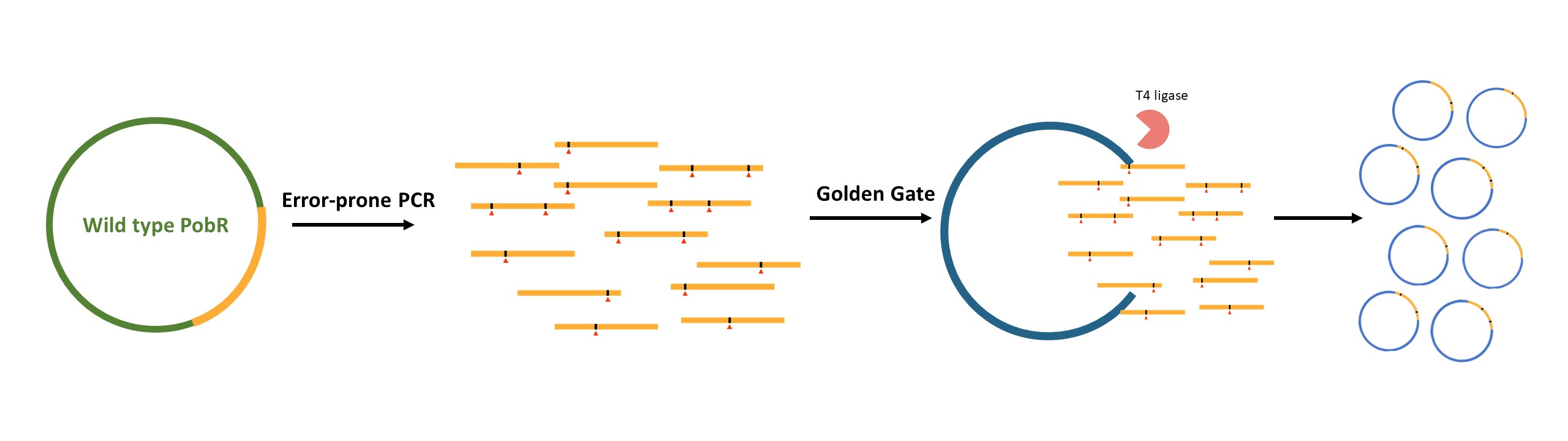
Figure 3. Schematic diagram of constructing the PobR mutant library.
Outcomes of the dual selections
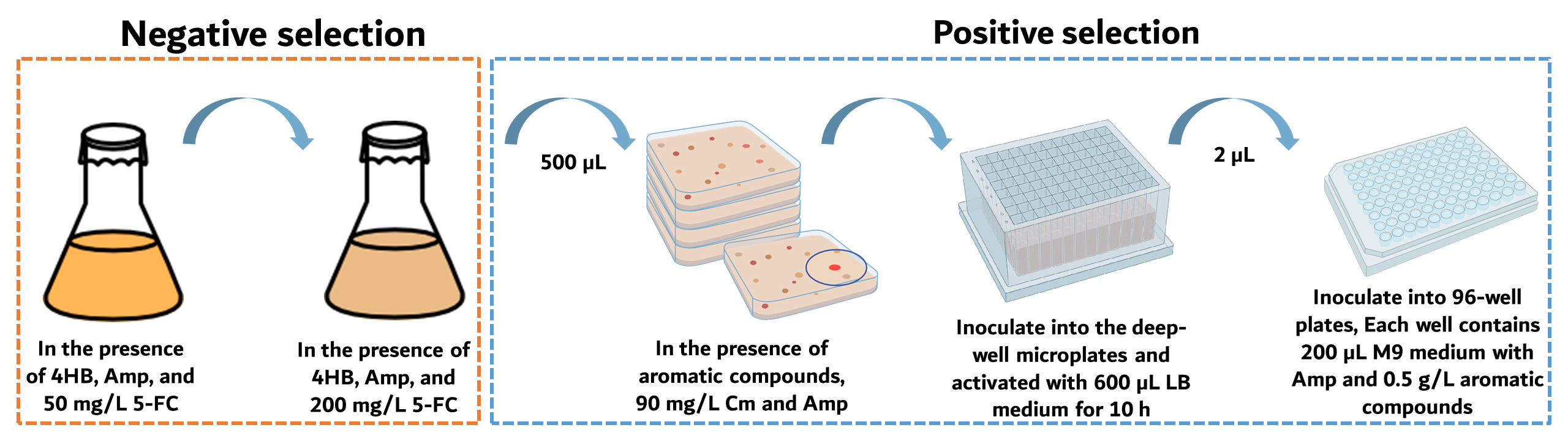
Figure 4. Flow chart of the dual selection process
As shown in Figure 4, we performed two rounds of the negative selection of the PobR mutants using 50 mg/L and 200 mg/L of 5-FC. By raising 5-FC concentration, we successfully eliminated pseudo-positive strains and those highly responsive to 4HB, increasing the effectiveness of the selection from 89% to 98%. In the positive selection, bacterial cultivation was conducted using the negative selection liquid medium individually supplied with different aromatic compounds, as well as chloramphenicol. Before starting, we evaluated the volume of the forward screening. The experimental procedure included aspirating 50 µl of the selected bacterial solution, adding it into 5,000 µl of LB medium (i.e., 1:100 dilution), and then taking 50 µl of the diluted bacteria to spread onto a LB-agar plate. Based on our calculation, the original density of the two-round selected bacteria was 450,000 CFU/mL. At least four plates were screened for each compound, with approximately 225,000 raw clones per plate.
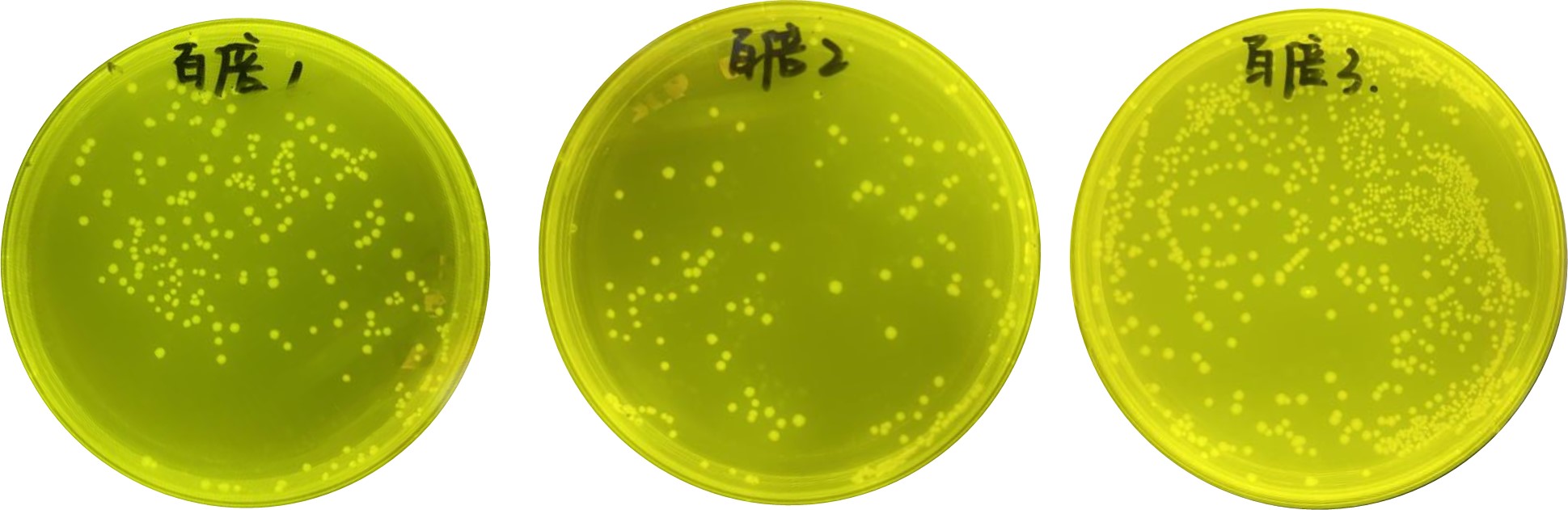
Figure 5. Clone calculation after passing two rounds of the negative selection in medium containing 50 mg/L and 200 mg/L 5-FC using the dilution coating method.
After preliminary characterization with 0.5 g/L of candidate ligands, we obtained several ligand-responsive strains, in which the fluorescence intensity was at least 1.5-fold higher than that of the negative control. Subsequently, we performed a second round of characterization experiments to individually examine their reactivity to each candidate ligand. After the second round of characterization, a total of nine potential PobR mutant biosensors were isolated, and their ligand response curves were plotted (Figure 6). Among them, the PobR mutants with relatively strong responses to HPP or PAA were selected.
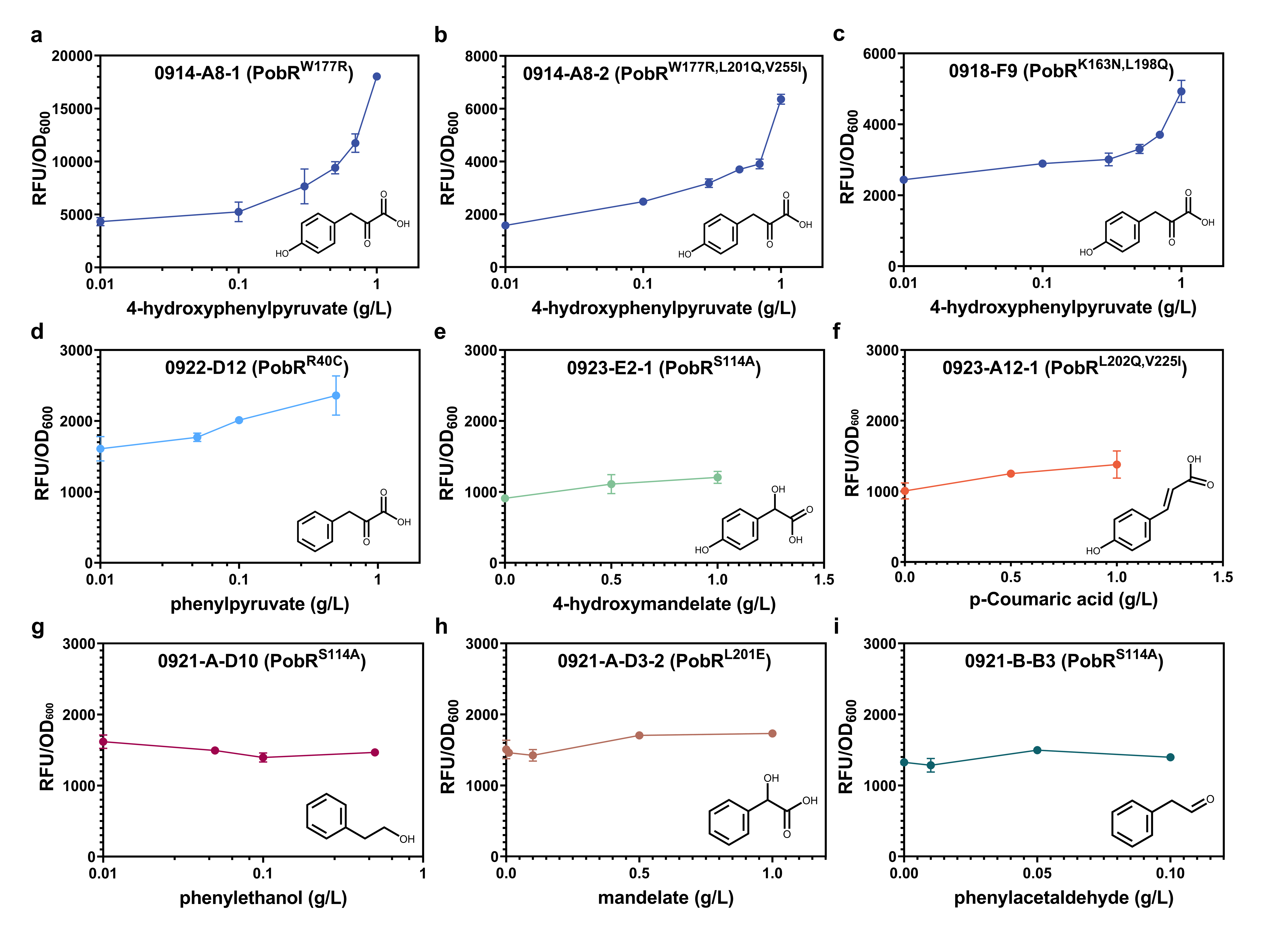
Figure 6. Characterization of the PobR mutants responsive to different aromatic compounds. (a) to (i). The fluorescent gene expression normalized by cell density (RFU/OD600) was plotted.
All nine potential biosensors obtained above were sequenced to identify the mutations in their primary sequences(Figure 7).
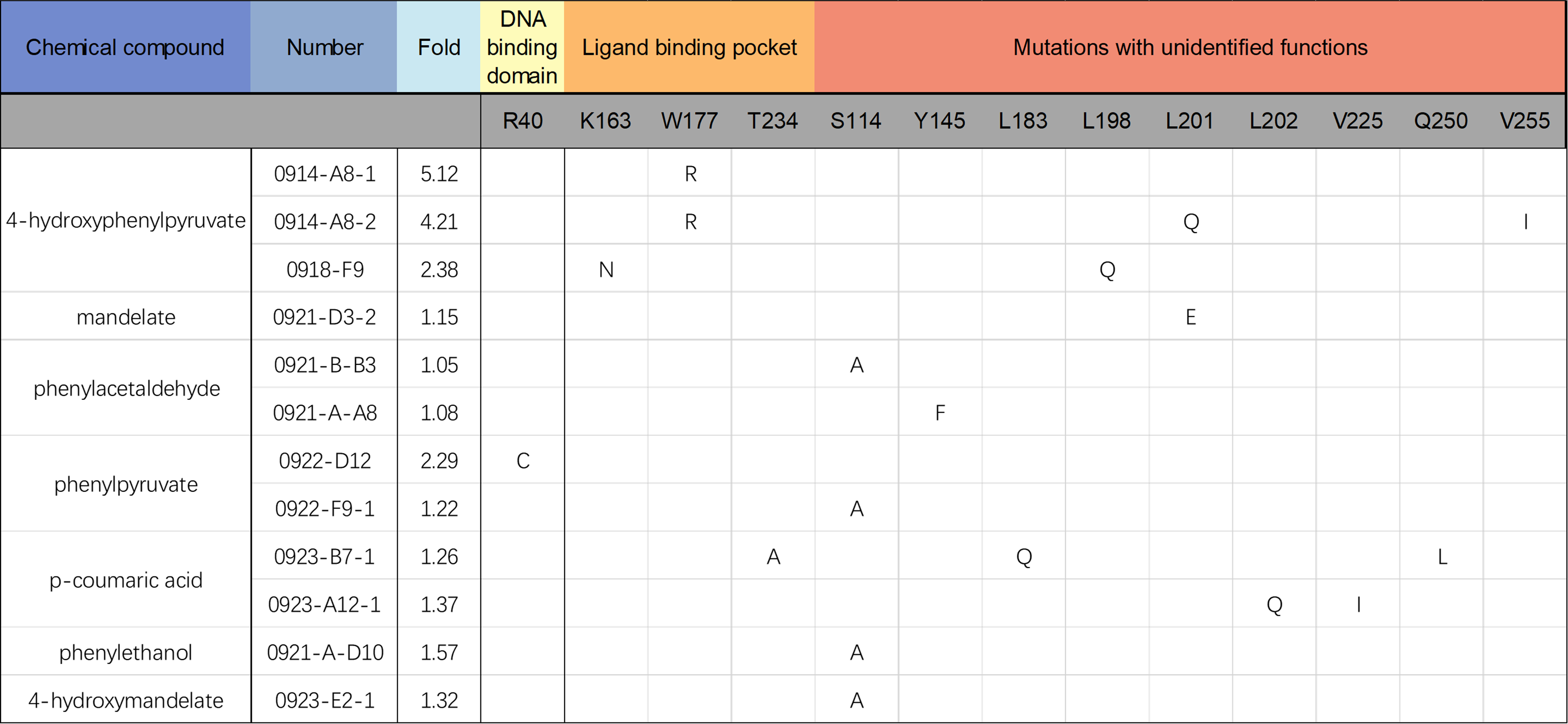
Figure 7. Amino acid substitutions in the transcriptional factor PobR mutants as biosensor candidates.
Analysis of the spatial locations of the mutated amino acids revealed that the residues 163, 177 and 234 of PobR are located near the ligand binding pocket, and the residue 40 is located in the DNA binding domain. The mutations of these amino acids likely exerted large impacts on the ligand recognition specificity of the PobR protein (Figure 8).
The remaining mutated residues (S114, Y145, L183, L198, L201, L202, V225, Q250 and V255) are distant from both the ligand binding and DNA binding domains of PobR, and thus the reasons for their altered binding affinity remain unclear. We speculated that the amino acid changes at these sites might indirectly alter the conformation of the ligand binding domain, or affect the dimerization of PobR. Interestingly, we discovered that four of the twelve variants harbored the same point mutation of S114A, indicating that the S-to-A substitution at the residue 144 could affect PobR ligand specificity to a great extent.
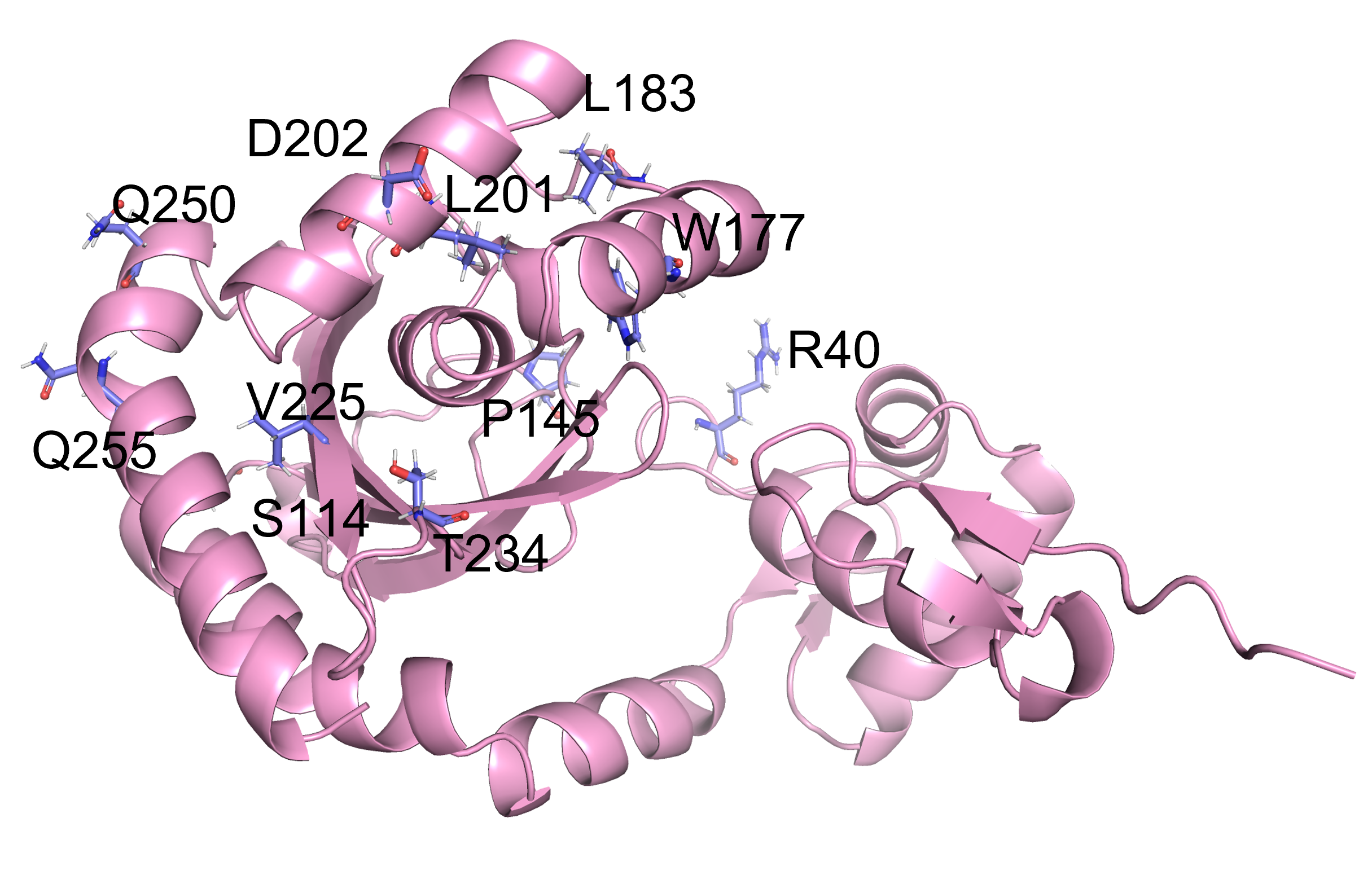
Figure 8. Analysis of the positions of mutated amino acids in PobR mutants using the PyMOL.
Among these PobR mutants, several of them were favorably responsive to HPP. As HPP concentration raised from 0.01 g/L to 1 g/L, their mCherry fluorescence showed a significant increase after 12 h of incubation. Among them, the best responsive sensor was the PobR mutant clone 0914-A8-1 with a point mutation of W177R, which showed a 5.1-fold increase of mCherry fluorescence signal in response to HPP (Figure 6). PobRW177R showed a significant change in specificity and a wider dynamic range (0.01-1.0 g/L), indicating decreased ligand binding affinity. We evaluated the specificity of PobRW177R to detect different aromatic compounds, and observed that 4HB could still activate it to enhance mCherry expression. Notably, although the PobRW177R mutant retained a 5.5-fold response to 4HB versus the condition without any ligand, its affinity to 4HB was significantly lower than that of PobRWT (28-fold reduction).
Additionally, at 0.5 g/L of PPA, another clone, PobRR40C, exhibited over 2 times of maximal induction by PPA compared to the condition without the ligand. Importantly, this PobR mutant showed marginal basal transcriptional activity. However, we also tested multiple additional aromatic compounds, including HMA, MA, p-Coumarins, etc., in the positive selection, and observed relatively weak responses to these compounds by some mutants. This could be due to the lack of capacity of our mutant library and insufficient selection rounds for these compounds.
Structure modeling and ligand docking
We used the SWISS-MODEL to simulate the three-dimensional structure of PobRW177R, and Autodock to simulate the interactions of HPP to PobRW177R and PobRWT. After analyzing the docking results, we found that HPP could form hydrogen bonds with I235 and R177 of PobRW177R, and hydrophobic interactions with L169, L165, L122, and V121 of PobRWT. In the surface model, R177 is spatially close to the HPP binding pocket of PobRW177R, while W177 is likely closer to a α-helix in PobRWT than that in the PobRW177R mutant. A comparison of hydrogen bonds revealed that R177 formed a hydrogen bond with HPP in the mutant, while W177 showed no interaction with HPP in PobRWT. Based on these analyses, we concluded that the W177R substitution allowed HPP to better interact with PobR.
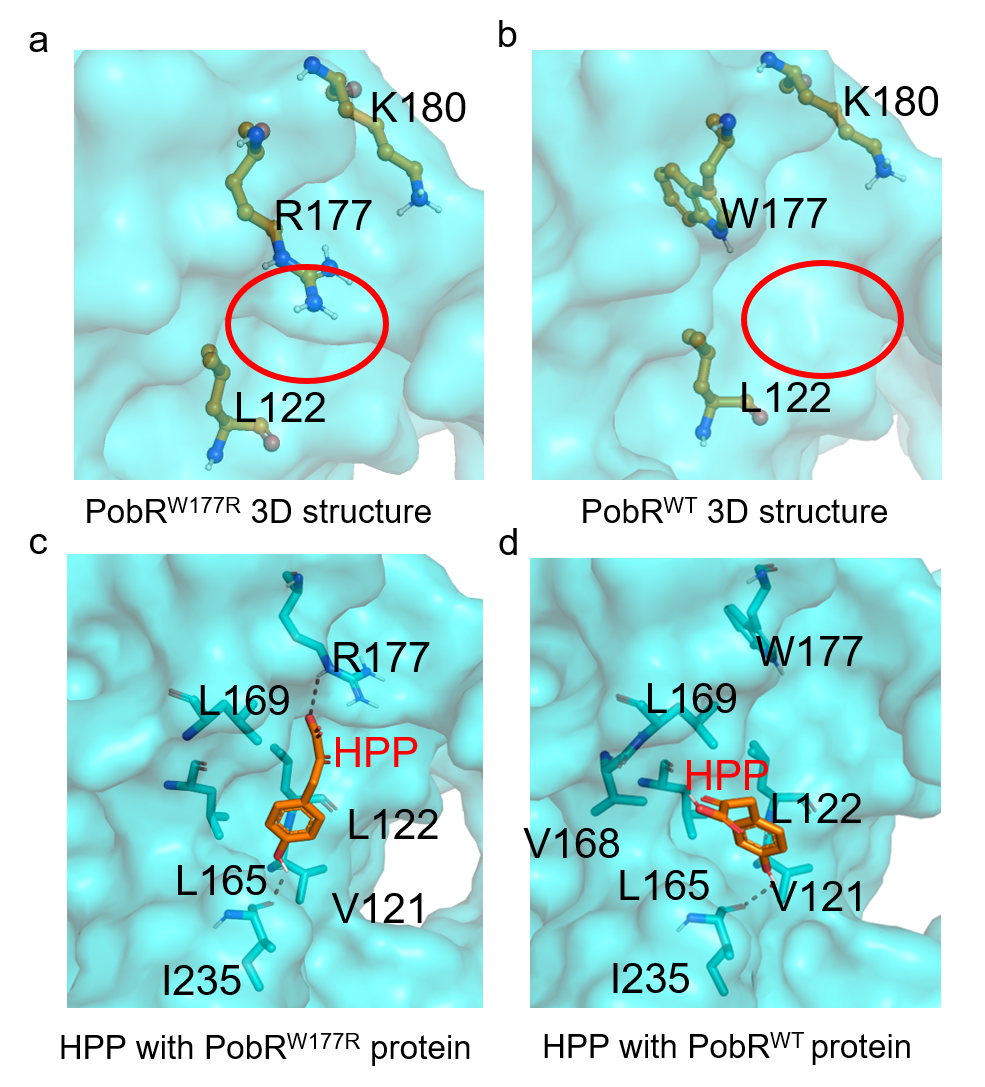
Figure 9. Structural analyses of PobRW177R and PobRWT proteins using the Autodock and PyMOL. Docking analyses generate binding simulation between HPP and the residues of PobR proteins.