Description
N-Boc-3-pyrrolidinol is an organic compound with the molecular formula C9H17NO3 and its structure is shown in the scheme. 1 (compound 2). (S)-1-Boc-3-hydroxypyrrolidine is a precursor in the synthesis of phosphodiesterase 10A inhibitor1. (R)-1-Boc-3-hydroxypyrrolidine is used for producing analogues of FTY7202.Whether S or R type, this optically active chiral alcohol is of great economic importance. For the preparation of optically active alcohols, enzyme-catalyzed biosynthesis is more attractive than traditional chemical synthesis because it is a highly selective and green approach3. Little work has been reported on the production of N-boc-3-pyrrolidinol using enzymes. Chiral alcohols can be obtained by keto reductases (KRED), which include aldol-keto reductases (AKR), short-chain alcohol dehydrogenases/reductases, and the medium-chain dehydrogenase family 4,5, while we focused on ethanol dehydrogenases, which are widely available in living organisms.
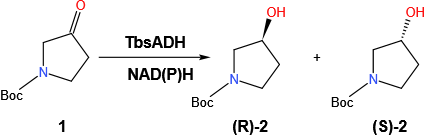
Scheme. 1. Asymmetric transformations to produce N-Boc-3-pyrrolidinol
Alcohol dehydrogenase (ADH) is found in large quantities in human and animal liver, plant and microbial cells and is a zinc-containing metalloenzyme with broad substrate specificity. As a key enzyme in the metabolism of the major short-chain alcohols in living organisms, it plays an important role in many physiological processes. Ethanol dehydrogenase is one of the stars of enzymatic industrial production 6. However, the optimum temperature of general ethanol dehydrogenase is around 40 °C, and its reaction efficiency decreases sharply after 45 °C, which limits the reaction rate of the chemical reactions it catalyzes to some extent7.
Philips et al. reported that a zinc ion, NAD+ (NADP+)-dependent ethanol dehydrogenase (TbsADH) derived from a thermophilic microorganism (Thermoethanolicus brockii) has good thermal stability and it can tolerate high concentrations of non-aqueous media, making TbsADH a very attractive industrial biocatalyst8. Wild-type TbsADH does not perform well in catalyzing the asymmetric reduction of pre-chiral ketones, and related works have been done to improve it9, but further improvement is needed in terms of catalytic efficiency and other aspects as far as the improvement results are concerned. We plan to modify TbsADH by means of directed evolution in the hope of obtaining a mutant with master selectivity and high reaction rate for catalytic N-Boc-3-pyrrolidone (NK) to N-Boc-3-pyrrolidinol (NA).
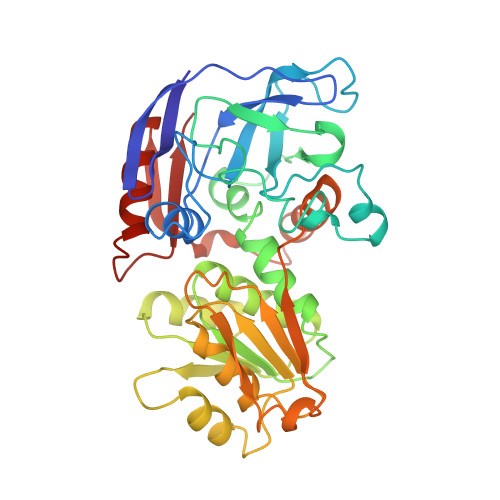
Fig. 1. Structure of TbsADH.(PDB)
Directed evolution mimics Darwinian evolution in a test tube and comprises iterative cycles of generating genetic diversity followed by screening/selection, which is a powerful method for creating biological macromolecules. Unlike natural evolution, whose goal is survival and reproduction, directed evolution is executed at much higher mutation and recombination rates to screen for the desired biological function. This approach not only yields a wealth of fundamental information about structure and evolution, but also allows the formation of useful biomolecules in research and industry. The general procedure of directed evolution includes two main steps: (1) gene diversification by random mutagenesis and/or gene recombination to generate a diverse library of variants; and (2) screening/selection to obtain variants with improved phenotypes. Irrational design typically employs methods such as error-prone PCR and DNA rearrangement that generate huge libraries of mutations, often requiring a great deal of time and effort. Rational design is performed based on the structure of the enzyme, where individual amino acids of the protein are mutated by the binding of the enzyme to the substrate, using physicochemical methods for assistance10.
But traditional directed evolution is often limited by depth and scale. In contrast, continuous directed evolution based on the replication system of the organism itself can save a lot of time and operations11. It undergoes directed evolution with minimal researcher intervention generation after generation. The experimental process is more "autonomous" than previous experiments. The methods of sequential directed evolution are now mature, and techniques such as phage-assisted sequential directed evolution(PACE), EvolvR, and Orthorep have been widely used12–14.
In our work, we tried to use both rational design and continuous directed evolution for the targeted modification of TbsADH with the aim of obtaining enzymes capable of catalyzing the efficient conversion of NK to NA.
But traditional directed evolution is often limited by depth and scale. In contrast, continuous directed evolution based on the replication system of the organism itself can save a lot of time and operations11. It undergoes directed evolution with minimal researcher intervention generation after generation. The experimental process is more "autonomous" than previous experiments. The methods of sequential directed evolution are now mature, and techniques such as phage-assisted sequential directed evolution(PACE), EvolvR, and Orthorep have been widely used12–14.
In our work, we tried to use both rational design and continuous directed evolution for the targeted modification of TbsADH with the aim of obtaining enzymes capable of catalyzing the efficient conversion of NK to NA.
Reference
1. Schwan, G. et al. Fluorine-containing 6,7-dialkoxybiaryl-based inhibitors for phosphodiesterase 10 A: synthesis and in vitro evaluation of inhibitory potency, selectivity, and metabolism. ChemMedChem 9, 1476–1487 (2014).
2. Fransson, R. et al. Design, Synthesis, and Antileukemic Activity of Stereochemically Defined Constrained Analogues of FTY720 (Gilenya). ACS Med. Chem. Lett. 4, 969–973 (2013).
3. Liu, X. et al. Characterization of a putative stereoselective oxidoreductase from Gluconobacter oxydans and its application in producing ethyl (R)-4-chloro-3-hydroxybutanoate ester. Mol. Biotechnol. 56, 285–295 (2014).
4. Patel, R. N. Enzymatic Synthesis of Chiral Intermediates for Drug Development. ChemInform 32, (2001).
5. Daußmann, T., Rosen, T. C. & Duenkelmann, P. Oxidoreductases and Hydroxynitrilase Lyases: Complementary Enzymatic Technologies for Chiral Alcohols. Eng. Life Sci. 6, 125–129 (2006).
6. Liu, J., Wu, S. & Li, Z. Recent advances in enzymatic oxidation of alcohols. Curr. Opin. Chem. Biol. 43, 77–86 (2018).
7. Kulishova, L. et al. Factors influencing the operational stability of NADPH-dependent alcohol dehydrogenase and an NADH-dependent variant thereof in gas/solid reactors. J. Mol. Catal. B Enzym. 67, 271–283 (2010).
8. Musa, M. M., Vieille, C. & Phillips, R. S. Secondary Alcohol Dehydrogenases from Thermoanaerobacter pseudoethanolicus and Thermoanaerobacter brockii as Robust Catalysts. Chembiochem Eur. J. Chem. Biol. 22, 1884–1893 (2021).
9. Sun, Z. et al. Catalytic Asymmetric Reduction of Difficult-to-Reduce Ketones: Triple-Code Saturation Mutagenesis of an Alcohol Dehydrogenase. ACS Catal. 6, 1598–1605 (2016).
10. Wang, Y. et al. Directed Evolution: Methodologies and Applications. Chem. Rev. 121, 12384–12444 (2021).
11. Rix, G. & Liu, C. C. Systems for in vivo hypermutation: a quest for scale and depth in directed evolution. Curr. Opin. Chem. Biol. 64, 20–26 (2021).
12. Esvelt, K. M., Carlson, J. C. & Liu, D. R. A system for the continuous directed evolution of biomolecules. Nature 472, 499–503 (2011).
13. Halperin, S. O. et al. CRISPR-guided DNA polymerases enable diversification of all nucleotides in a tunable window. Nature 560, 248–252 (2018).
14. Ravikumar, A., Arzumanyan, G. A., Obadi, M. K. A., Javanpour, A. A. & Liu, C. C. Scalable, Continuous Evolution of Genes at Mutation Rates above Genomic Error Thresholds. Cell 175, 1946-1957.e13 (2018).
Next :Design Page